Lot 777
Mouse iPSC generated with porcine reprogramming factors as a model for studying the effects of non-silenced heterologous transgenes on pluripotency
Petkov SG1, Glage S2, Niemann H3
Lot Polish Airlines 777
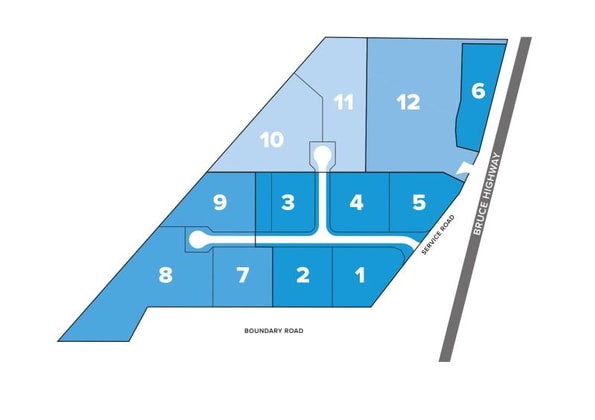
Lot 777; Ferdinand Kriwet - Textmobil. Ferdinand Kriwet Textmobil 1970/1971 Silkscreen on movable plexiglass panes infront of plastic. Lot 777 $ 54,950. 9705 Lake Bess Rd Lot # 777 7th Street. New Refrigerator, Like Brand new. New paint & Floors, High. Lining up combinations of winning symbols is incredibly entertaining and wonderfully rewarding. With slots games, you truly have carte blanche to place bets tailored to your bankroll. In fact, at 777 you can even practice your favourite slots online for free before you register, deposit and claim your welcome bonus.
Author Names in full: Stoyan G Petkov1, Silke Glage2, Heiner Niemann3
1 German Primate Center, Goettingen, Germany, 2 Hannover Medical School, Hannover, Germany, 3 Institute for Farm Animal Genetics (FLI), Neustadt, Germany
doi:10.46582/jsrm.1301004
Abstract
Mouse somatic cells can be reprogrammed to pluripotency by the ectopic expression of four pluripotency transcription factors, Oct4, Sox2, c-myc, and Klf4. Usually, silencing of the exogenous reprogramming factors is considered to be essential for complete reprogramming and differentiation. In the vast majority of studies, murine pluripotency transcription factor sequences have been used for the reprogramming of mouse fibroblasts to induced pluripotent stem cells (iPSC). The effectiveness of xenogeneic transcription factors in miPSC generation has not yet been investigated in detail. Here, we evaluated transposon-based vectors with four porcine pluripotency factors for their ability to reprogram mouse fetal fibroblasts (MEFs) harboring an Oct4-EGFP reporter construct to pluripotency. Additionally, we examined the effects of the non-silenced heterologous transgenes on the expression levels of key endogenous pluripotency markers and the differentiation capacities of the miPSC. Within 8 days of transfection with porcine reprogramming transcription factors the MEFs acquired typical compact miPSC morphology and upregulated expression of endogenous Oct4 and other critical pluripotency genes. Consequently, the transgenes under the control of the TetO promoter became silenced, while the CAG-controlled constructs were expressed throughout the period of culture. Despite the continuous transgene expression, the CAG-miPSC showed normal morphology and were capable of differentiation into the three primary germ layers in vitro and in vivo. However, the expression levels of important endogenous pluripotency markers, Klf4, c-myc, Rex1, and Utf1, were significantly lower in CAG-miPSC compared with TetO-miPSC with silenced reprogramming cassettes. Surprisingly, the endogenous Oct4 and Sox2 expression levels were not affected by the residual transgene expression. Our results suggest that porcine reprogramming transcription factors are suitable for production of miPSC, but silencing of the heterologous transgenes may be necessary for complete reprogramming to pluripotency.
Keywords: iPSC; Reprogramming; Silencing
Lot 777
Introduction
The fate of a terminally differentiated somatic cell can be reversed by ectopic expression of pluripotency-related factors, as demonstrated by Takahashi and Yamanaka[1]. In experiments that have since revolutionized the stem cell field, it was demonstrated that elevated expression of only four proteins such as Oct4, Sox2, c-myc, and Klf4 leads to upregulation of endogenous pluripotency transcription factors and ultimately to acquisition of pluripotent characteristics by the reprogrammed cells[2,3]. To underscore the difference to alternative reprogramming methods (e.g. by somatic cell nuclear transfer, cell extracts, etc.), the resulting stem cells have been termed “induced pluripotent stem cells” (iPSC). During the reprogramming process, the balance between the expression levels of the endogenous pluripotency genes and the transgenes is thought to be critical for the success of the reprogramming of somatic cells to iPSC, with the epigenetic silencing of the retroviral transgenes coinciding with the up-regulation of the endogenes[4]. It has been shown that over-expression of Oct4 in mouse embryonic stem cells (mESC) leads to mesodermal differentiation[5], while elevated Sox2 expression causes neuroectodermal specification[6]. Consequently, the net concentration levels of Oct4 and Sox2 in the iPSC would need to be maintained within a narrow range characteristic for ESC. In support of this hypothesis, it was demonstrated that following upregulation of the corresponding endogenous pluripotency factors, the transgenes became gradually silenced in successfully reprogrammed iPS cells[7,8]. Persisting transgene expression results in perturbations in the reprogramming process, usually leading to partially reprogrammed states (Class I iPSC), as shown by Mikkelsen and co-workers[9]. Residual transgene expression restricted the differentiation capacities of human iPSC[10] and affected epigenetic signatures and gene expression levels of miPSC compared with mESC[11]. Moreover, in a recent report, miPSC produced with transposon-delivered transgenes that were continuously expressed by supplementation with doxycycline (DOX) were reversed to an alternative pluripotency state (F-state), characterized by aberrant colony morphology and dependency on the continuous transgene expression for their maintenance[12].
It has been convincingly demonstrated that the differentiation capacities of murine iPSC lines with constitutive expression of non-silenced reprogramming factors are limited. However, conflicting results were reported by different research groups. The miPSC produced in the first attempts of Takahashi and Yamanaka[1] did not silence the reprogramming factors and were not able to differentiate and form chimeras. Similarly, transgene-expressing miPSC were unable to differentiate and upon injection into immunodefficient mice formed homogenous tumors, consisting of undifferentiated cells expressing high levels of Oct4[13]. On the other hand, the use of a constitutively expressed reprogramming cassette led to miPSC with normal ESC-like characteristics, which were capable of forming the three primary germ layers in teratomas[14]. However, these cells also exhibited gene expression differences compared with ESC and excision of the loxP-flanked transgenes resulted in miPSC with complete pluripotency characteristics.
While the vast majority of miPSC described in the literature have been generated with murine pluripotency transcription factors, there have been very few reports on the use of transgenes from other species. Only one study has reported the use of human reprogramming factors to successfully reprogram mouse fibroblasts to iPSC[15]. In addition, the silencing of non-murine reprogramming factors as well as the effects of the non-silenced xenogeneic transgenes on the expression of endogenous pluripotency genes and its relationship with differentiation of miPSC have not been yet investigated. Here, we explored the possibility to use porcine transcription factors for the reprogramming of mouse embryonic fibroblasts (MEFs) to pluripotency. Established miPSC lines constitutively expressing the four porcine Yamanaka factors (pOCT4, pSOX2, pc-MYC, and pKLF4) under the control of the ubiquitous CAG promoter were characterized for pluripotency and their differentiation potential together with the expression levels of master pluripotency genes were compared with those of miPSC produced either with a silenced or CRE recombinase-excised reprogramming cassette.
Materials and methods
Unless specified otherwise, the chemicals and kits used in this study were purchased from Sigma-Andrich.
Derivation of mouse fetal fibroblasts for feeder cells and reprogramming
Outbred NMRI mice were purchased from Harlan Laboratories (Horst, Netherlands) and were used to establish MEFs for feeder layers. C57BL/6 mice harboring Oct4-EGFP pluripotency gene reporter (OG2) were kindly provided by Prof. Hans Schoeler (Max-Planck-Institute for Molecular Biomedicine, Muenster, Germany) and were used to generate MEFs for reprogramming to iPSC. For the generation of MEFs, pregnant mice were sacrificed at day 13-13.5 of gestation, fetuses were dissected and the heads, livers, mesonephros, and hearts were removed. The remaining parts, including limb buds and body wall were minced with a scalpel blade, incubated in trypsin/EDTA solution for 20 min at 37°C and disaggregated to single cells and clumps by vigorous pipetting after adding warm culture medium. The culture medium used was high glucose DMEM (Lonza), supplemented with 15% fetal bovine serum (FBS) (Gibco, Lot 41Q2035K), non-essential amino acids (GE Healthcare), sodium pyruvate, 2 mM Glutamine (GE Healthcare), and 0.1 mM 2-mercaptoethanol. Feeder cells were produced by treating MEFs from NMRI mice with 10 µg/ml mitomycin C for 2 hours.
Reprogramming expression vectors
The construction of the Sleeping Beauty (SB) transposon plasmids with either the Tet-On (TetO) promoter (SB-TetO-pOSMK-IRES-Tomato, used together with SB-CMV-rTA-IRES-Neor) or with the constitutive CAG promoter (SB-CAG-pOSMK-IRES-Tomato) for expression of the porcine OCT4, SOX2, c-MYC, and KLF4 have been described previously[16]. To create a CRE-excisable version, the CAG promoter and the polyadenylation signal were amplified using loxP-containing forward or reverse primer, respectively, and these modified sequences were used to replace the originals by standard DNA cloning techniques, thus generating a loxP-flanked CAG-pOSMK-IRES-Tomato-pA cassette. For efficient integration into MEFs, the plasmid with a hyperactive version of the SB (SB100x) was co-transfected with each of the reprogramming transposons. The SB transposon vector backbone and transposase expression plasmids were provided by Dr. Zoltan Ivics (Paul-Ehrlich-Institute, Langen, Germany).
Mouse iPSC generation and culture
Three different MEF lines from C57BL/6-OG2 mice were electroporated at passages 3-4 with the SB transposon and transposase plasmids as described by Petkov et al. [16] and plated on gelatinized T75 flasks. The cells were cultured in MEFs culture medium for 2-3 days and then were then split 1:3-1:4 to T75 flasks with mitotically inactivated MEF feeders and the medium was supplemented with 1000 U/ml ESGRO murine leukemia inhibitory factor (mLIF) (Millipore). When vectors with the TetO promoter were used, the medium was supplemented with 5 µg/ml DOX. The OCT4-EGFP-positive colonies with ESC-like morphology were manually picked at day 10-11 post-transfection, disaggregated with Trypsin/EDTA, and cultured individually on mytomycin C-inactivated MEFs to establish individual cell lines. The established miPSC were regularly split every 3-4 days at ratio 1:15-1:20 by trypsinization and maintained on fresh feeders in ESGRO medium for over 70 passages. For generation of transgene-free miPSC, the miPSC harboring the “floxed” version of the reprogramming transposon were transfected with SB-CAG-CRE-IRES-Puror vector using Lipofectamine 2000 (Life Sciences) as instructed by the manufacturer and selected with culture medium supplemented with 3 µg/ml puromycin.
Alkaline phosphatase (AP) staining and immunocytochemistry
Lot Airlines 777
The cultured were fixed with 4% Formalin solution for 5 min (for AP) or 15 min (antibody staining) and washed with PBS prior to further processing. For AP staining, a solution containing 1 mg/ml Fast Red and 0.4 mg/ml Naphtol-BI-Phosphate in AP-buffer (100 mM NaCl2, 100 mM Tris (pH 9.5), and 50 mM MgCl2) was applied to the fixed wells. The AP-positive cells were discerned by the formation of insoluble red-brown precipitate. The presence of SSEA-1, SSEA-3, SSEA-4, TRA-1-60, and TRA-1-81 expression in miPSC was assessed by incubation with primary antibodies (Thermo; Cat. numbers 41-1200, MA1-020X, 41-4000, 41-1000, 41-1100, respectively), diluted in PBS with 2% Knockout Serum Replacement (KSR) (Invitrogen) at concentration 10 ng/ml for 1 hour, followed by washing with PBS and incubation with the secondary peroxidase - conjugated antibody. For staining of neuronal-like cells, the anti-PGP 9.5 primary antibody (DACO) was used in a similar protocol. The expressed proteins were visualized after incubation with peroxidase substrate from AEC staining kit.
Reverse transcription, PCR, and real-time PCR
For RNA extraction, the miPSC cultures were washed 3 times with Ca- and Mg-free PBS and incubated in the same solution for 15 minutes at 37 degrees C. The miPSC colonies were detached from the feeders by gentle pipetting, collected in a 15 ml centrifuge tube and allowed to settle on the bottom of the tube for 10 min. The supernatant containing single cells (presumably mostly feeder MEFs) was carefully removed, the pellet was re-suspended in PBS and the colonies were pelleted by centrifugation, lysed in TRI reagent (Ambion), and total RNA was extracted as instructed by the manufacturer. Reverse-transcription was carried out with MuLV Reverse Transcriptase (Applied Biosystems). Polymerase chain reaction was performed using 50 ng reverse transcribed RNA per 25 µl reaction volume using Platinum Taq Polymerase (Invitrogen).Real-time relative quantitative analysis was run on the ABI 7500 Fast System using SYBR Green Master Mix (Applied Biosystems). The primers used for PCR and real-time PCR analysis of endogenous mouse pluripotency markers Oct4, Sox2, Nanog, c-myc, Klf4, Rex1, and Utf1 as well as for the differentiation markers alpha fetoprotein (Afp), Sox17, Gata4, Gata6, cardiac troponin, myosin heavy chain (MHC), nestin, β-III tubulin, and Pax6 are shown in Table S1. The primers used for the amplification of the mouse endogenous pluripotency genes were specific only for the mouse cDNA sequences and did not amplify the pig pOSMK cassette (results not shown). The primers used for the amplification of the pOSMK-IRES-Tomato construct were a forward primer binding at the end of KLF4 and a reverse primer binding to IRES (shown in Table S1). The data from the relative quantitative analysis were analyzed with GeneEx software (bioMCC). Statistically significant differences were determined by Student’s t-test performed by the same computer program.
Karyotyping
Three lines each from TetO- and CAG miPSC at passages 58-60 were treated with Demecolcine solution (Sigma, D1925) added at 100 µl/10ml culture medium for 35 min. The cells were harvested by trypsinization, incubated in hypotonic solution (0.28% KCl + 0.25% Na Citrate) for 25 min at 37˚C, and fixed in cold (-20˚C) methanol: acetic acid (3:1) fixative for 1 hour. Metaphase spreads were produced by dropping 50 µl drops onto glass slides placed at a slight angle over steaming water bath (95˚C) and waiting 1 min before removal and air drying. The metaphase spreads were stained with 2% Giemsa solution. The metaphase chromosomes of at least 15 metaphase spreads per cell line were counted to determine whether the cells had normal karyotype.

Embryoid body (EB) formation
Mouse iPSC colonies were separated from the feeder as described above in subsection 2.5, disaggregated to single cells with trypsin, and suspended in basic culture medium without ESGRO. “Hanging drops” were then produced by making 25 µl drops on the inner side of a 10 mm Petri dish lid (containing 2000 cells/drop) and placing the lid back on the dish filled with PBS (to prevent drying of the drops). After 5 days in “hanging drops” culture, the emerging embryoid bodies were plated in gelatin-treated 6-well plates and cultured further for 15 days with changes of the medium every 3-4 days. For neuronal differentiation, the attached EBs were cultured in N2 medium (DMEM/F12 supplemented with sodium pyruvate, amino acids, penicillin-streptomycin, and N2 supplement (Thermo)).
Teratoma formation
Prior to conducting the animal experiments, permission was obtained from the local animal welfare authority (LAVES).
Nude immunodeficient mice were produced by mating outbred NMRI strain parents. A total of 13 nude mice (7 mice/TetO group and 6 mice/CAG group) were injected each with 1 x106 putative iPSC derived from reprogramming with the porcine vectors subcutaneously in the right flank. The mice were observed daily for 3 weeks. After reaching the critical size of the tumour (1 cm), the animals were sacrificed. Biopsies were taken for DNA/RNA extraction, and tissues were fixed in neutral buffered 4% formalin not exceeding 48 h, dehydrated (Shandon Hypercenter, XP) and subsequently embedded in paraffin (TES, Medite). Sections (2-3 μm thick, microtome Reichert-Jung 2030), were deparaffinized in xylene and H&E stained according to standard protocols. The morphological evaluation for the presence of the three germ layers (Axioskop 40, Zeiss microscope) was performed by a trained pathologist and representative microphotographs were taken (AxioCam MRc, Zeiss).
Results
Reprogramming to pluripotency
Transfected MEFs cultured on inactivated feeders formed O4-EGFP-positive colonies with typical miPSC-like morphology within 7-8 days post-transfection (Figure 1A, D). A number of granular colonies consisting of round, highly refractory cells were also observed.
Figure 1 –Mouse iPSC generated with Sleeping Beauty transposons carrying porcine reprogramming factors. A) TetO-miPSC at day 8 post-transfection with typical miPSC morphology. B) Oct4-EGFP reporter expression in the colony in image A. C) Silenced pOSMK-IRES-Tomato expression except in some cells in image A. D) CAG-miPSC at day 8 post-transfection. The colony has compact miPSC-like morphology. E) Expression of Oct4-EGFP reporter in the colony shown in image D. F) Expression of non-silenced pOSMK-IRES-Tomato construct in the colony from image D. G) CAG-miPSC at P.22. The colonies maintained compact miPSC morphology. H) Oct4-EGFP reporter expression in the colonies shown in image G. I) Expression of pOSMK-IRES-Tomato in the colonies shown in image G. (All scale bars = 50 µm).
There was no significant difference in the timing of EGFP-positive colony appearance between the different groups where transposons with TetO or CAG were used. In most miPSC colonies produced with the TetO promoter, the Tomato fluorescence (indicating pOSMK transgene expression) disappeared completely from the cells by day 10-11 of primary cultures (Figure 1C), and was no longer detectable in the presence of DOX, indicating successful transgene silencing. The colonies that retained the Tomato fluorescence were not picked. All miPSC produced with CAG promoters retained weak Tomato expression throughout the entire time of culture (>70 passages) (Figure 1 F, I). All of the picked colonies (12 colonies/transfected MEF line) formed clonal lines when cultured in individual wells; however, some TetO-lines showed signs of differentiation after 3-4 passages and were discarded. Five lines from each group were chosen for long-term culture.
Expression of master pluripotency markers
The pluripotency-related surface antigens AP and SSEA-1 were expressed in both groups, while SSEA-3, SSEA-4, TRA-1-61, and TRA-1-80 were not detected (Figure 2). RT-PCR confirmed expression of the endogenous Oct4, Sox2, Nanog, c-myc, Klf4, Rex1, and Utf1 in both CAG- and TetO-miPSC (Figure 3A). Expression of the transgenes was detected only in the CAG group (Figure 3A). To determine whether there was a difference in the expression levels of endogenous pluripotency genes between the CAG and TetO groups, we carried out real-time relative quantitation analysis. While there was no significant difference in the expression of Oct4, Sox2, and Nanog (P>0.05), the expression levels of c-myc, Klf4, Rex1, and Utf1were significantly higher in the TetO group compared with the CAG-miPSC (p<0.05) (Figure 3B). When the pOSMK cassette was removed from the CAG group by CRE expression, there was no significant change in the expression levels of the endogenous pluripotency genes (p>0.05), except for Utf1, which was up-regulated in the CRE-treated cells (p<0.01) (Figure 3C).
Figure 2 –Expression of surface pluripotency markers in CAG-miPSC at P. 22. A) AP. B) SSEA-1. C) SSEA-3. D) SSEA-4 E) TRA-1-60. F) TRA-1-81. (Scale bars = 50 µm).
Figure 3 –Gene expression in miPSC produced with porcine reprogramming factors. A) RT-PCR analysis of major endogenous pluripotency genes and transgenes in miPSC. B) Real-time relative quantitative analysis of expression level differences between TetO- and CAG-miPSC. C) Real-time relative quantitative analysis of expression level differences between miPSC with CRE-excised and still expressed transgenes. Statistically significant differences (p< 0.05) are indicated with asterisks.
Karyotyping
Each of the 6 examined miPSC lines had 20 chromosome pairs and no aberrations from the normal diploid karyotype were found (Figure S1).

In vitro and in vivo differentiation
The cells from both experimental groups formed EBs within 5 days of differentiation culture in “hanging drops”. Upon attachment on gelatin-treated plastic surfaces, the cells expanded and spontaneously differentiated into epithelial-like cells (Figure 4A, endoderm), neuronal-like cells that reacted positively with the anti-PGP 9.5 antibody (Figure 4A, ectoderm), and rhythmically contracting cardiac myocytes (Figure 4A, mesoderm; Video S1). The expression of differentiation markers Afp, Sox17, Gata4, Gata6, MHC, troponin, nestin, β-III tubulin, and Pax6 in EB was confirmed by RT-PCR (Figure 4B).
Figure 4 –In vitro and in vivo differentiation of miPSC produced with porcine reprogramming factors. A) In vitro differentiation of CAG-miPSC: epithelial-like cells (endoderm) (scale bar = 50 mm), neuronal-like cells positive for PGP 9.5 (ectoderm) (scale bar = 20 mm), and spontaneously contracting cardiac myocytes (mesoderm), indicated with arrows (scale bar = 50 mm). (For additional data on cardiomyocyte contraction, see Supplementary Video 1). B) Expression of differentiation markers in EB outgrowths by RT-PCR.
When injected into immunodefficient mice, 3/7 (43%) mice injected with cells from the TetO group and 6/6 (100%) mice injected with miPSC from the CAG group formed teratomas within 3 weeks post-injection. Histological analysis of the teratomas revealed presence of the three germ layers in teratomas produced with miPSC from both the TetO and CAG groups (Fig. 5). We also visually examined fresh biopsies for the presence of EGFP fluorescence as indication of undifferentiated cells and found EGFP-positive cells in one of the CAG-miPSC-derived teratomas. The expression levels of key endogenous pluripotency genes, the pOSMK transgenes, and main differentiation markers were compared between miPSC from the CAG group and the teratomas by real-time relative quantitative analysis. As expected, Oct4, Nanog, Rex1, and Utf1 were significantly down-regulated, while differentiation markers Afp, Sox17, Gata4, Gata6, Pax6, and Nestin were significantly up-regulated in teratomas (all p<0.01) (Fig. 6). There was no significant difference in the expression levels of Sox2 and Klf4, while c-myc was significantly higher expressed in teratomas (P<0.05). These results were similar to the TetO group, except for c-myc, which did not show a significant difference (results not shown). Expression of the reprogramming pOSMK cassette could still be detected in two teratomas generated with CAG-miPSC, but the expression levels were low, as suggested by real-time PCR threshold values higher than 33, so relative quantitative analysis could not be reliably completed.
Figure 5 – In vivo differentiation of miPSC in teratomas. TetO-miPSC injected into nude mice formed ciliated epithelium with mucous- producing cells (A), neuronal tubes (B), and connective tissue (C) (scale bars = 50 µm). CAG-miPSC differentiated into ciliated epithelium (D), neural tubes (E), cartilage and bone (F), epithelial cysts (G), keratinized epithelium (H), and connective tissue (I). (Scale bars = 100 µm).
Figure 6 –Real-time relative quantitation analysis of gene expression in CAG-miPSC and teratomas. Statistically significant differences are indicated with asterisks.
Discussion
Here, we investigated whether four porcine reprogramming transcription factors were able to successfully reprogram MEFs to pluripotency. The amino acid sequences for Oct4, Sox2, c-myc, and Klf4 are evolutionary highly conserved, although not fully identical between mouse and pig (88%, 98%, 93%, and 94%, respectively; amino acid sequence alignments are shown on Figure S2). Thus, it was necessary to confirm that the porcine proteins can in fact reprogram somatic cells across species. As expected, the MEFs changed proliferation and morphology and upregulated endogenous OCT4 expression, as demonstrated by the presence of EGFP fluorescence and subsequently confirmed by RT-PCR. The picked colonies proliferated robustly and established cell lines with typical compact miPSC morphology and expression of key pluripotency markers such as AP, SSEA-1, Oct4, Sox2, Nanog, Klf4, Rex1, and Utf1. At the same time, the TetO-promoter-controlled transgenes were silenced, even in the presence of DOX. This result is in contrast with other reports, where transposon-delivered transgenes were not silenced by the reprogrammed cells if the culture medium was continuously supplemented with DOX[12, 17]. A possible explanation for our results is that the porcine transgenes are more efficiently recognized and silenced by the miPSC. Silencing of the reprogramming transcription factors has been described as an essential prerequisite for successful reprogramming[2, 7- 9]. Thus, porcine reprogramming factors may be useful for the production of miPSC, particularly where significant nucleotide sequence differences between the transgenes and the endogenous pluripotency genes are desired.
Another interesting question of this study was whether the mouse cells would balance the expression levels of key endogenous pluripotency genes with the non-silenced porcine transgenes. We obtained cell lines with silenced (TetO-regulated) and non-silenced (CAG-regulated) reprogramming cassettes, which enabled us to address this question by comparing the expression levels of endogenous pluripotency genes between the two groups. Surprisingly, we found no significant differences in the expression levels of endogenous mouse Oct4 and Sox2 between the cells with silenced vs. expressed transgenes. Additionally, the expression levels of these genes did not change even after the transgenes were removed by expression of CRE recombinase. These results suggest that the porcine transgenes did not participate in the regulation of endogenous Oct4 and Sox2 expression after the initial induction. Moreover, none of the cells from the CAG-group showed any signs of differentiation that would be expected from elevated total Oct4 and Sox2 expression levels. A possible explanation for this outcome could be the very low expression levels of the transgenes, as suggested by our real-time PCR analysis. Another possibility is that the specifying signals ensuing from elevated total Oct4 and Sox2 levels had balanced each other, preventing differentiation into any of the germ layers. On the other hand, the expression levels of the endogenous c-myc, Klf4, Rex1, and Utf1 were significantly lower in cell lines with non-silenced transgenes compared with the TetO group. Excision of the reprogramming cassette by CRE-recombinase resulted in significant upregulation of Utf1, but failed to upregulate the rest.
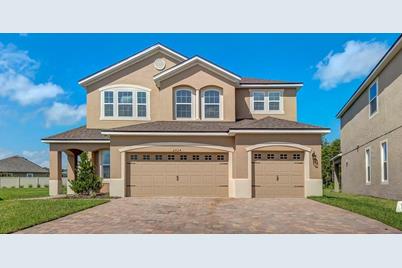
Despite the deviations in the expression of important pluripotency markers, the cell lines with residual expression of the four Yamanaka factors were capable of differentiation into derivatives of the three primary germ layers in vitro and in vivo (by forming fully differentiated teratomas) similar to the TetO- group. When we examined the expression levels of pluripotency and differentiation-related genes in teratomas and iPSC lines, we found that, as expected, the pluripotency markers were down-regulated while the differentiation markers were up-regulated, suggesting normal differentiation process in the CAG-iPSC.
Unlike the miPSC in an alternative state of pluripotency (F-state) described recently[12], our miPSC lines with non-silenced reprogramming factors have compact ES-like morphology and do not depend on the expression of the transgenes for their maintenance; therefore, they do not necessarily fall into an alternative pluripotency state category. Nevertheless, it has been shown that even subtle aberrations in miPSC with residual transgene expression could impair the full developmental competency of miPSC[11, 14]. The lower expression of important pluripotency markers such as Rex1, Utf1, and Klf4 in CAG-miPSC suggests that this may also apply for miPSC produced with non-silenced porcine transcription factors. In future work, the cells will be tested for chimera formation to ascertain whether they are fully competent iPSC.
Conclusions
In conclusion, we successfully established miPSC using four porcine reprogramming transcription factors and examined the effects of the non-silenced transgenes on the expression levels of key endogenous pluripotency markers. The residual expression of the transgenes affected expression levels of important pluripotency genes such as c-myc, Klf4, Rex1, and Utf1. These results suggest that porcine pluripotency transcription factors are suitable for reprogramming of mouse somatic cells to pluripotency, but epigenetic silencing of the xenogeneic transgenes may be necessary for complete acquisition of pluripotency.
Potential Conflicts of Interests
None
Lot 777
Acknowledgements
Dr. Wilfried Kues (Institute of Farm Animal Genetics, Friedrich-Loeffler-Institute, Mariensee, Neustadt, Germany) for the injection of the immunodefficient mice. Dr. Hans Schoeler (Max-Planck-Institute for Molecular Biomedicine, Muenster, Germany) for providing the OG2 reporter mice. Dr. Zoltan Ivics (Paul-Ehrlich Institute, Langen, Germany) for providing the SB transposon backbone and the SB transposase expression plasmid. Dr. Poul Hyttel (University of Copenhagen, Copenhagen, Denmark) for allowing us to use and modify the transposon plasmid which was generated by Dr. Stoyan Petkov in his laboratory.
Sponsor / Grants

German Research Foundation (Deutsche Forschungsgemeinschaft), Grant Ni 256/ 32-1.
Additional Information
Supplementary Information accompanies this article.
To view the Supplementary figures and table click here
To download the Supplementary Video click here
Abbreviations used in the article
Afp: | Alpha-fetoprotein |
AP: | Alkaline Phosphatase |
CAG: | Synthetic Promoter containing the (C) cytomegalovirus early enhancer element, (A) the promoter, the first exon and the first intron of chicken beta-actin gene, and (G) the splice acceptor of the rabbit beta-globin gene |
DMEM: | Dulbecco’s Modified Eagle’s Medium |
DOX: | Doxycycline |
EGFP: | Enhanced Green Fluorescent Protein |
FBS: | Fetal Bovine Serum |
iPSC: | Induced Pluripotent Stem Cells |
mESC: | Mouse Embryonic Stem Cells |
Klf4: | Kruppel-Like Factor 4 |
MEFs: | Mouse Embryonic Fibroblasts |
MHC: | Myosin Heavy Chain |
Oct4: | POU Domain, Class 5, Transcription Factor 1 |
Pax6: | Paired Box Protein 6 |
PBS: | Phosphate-Buffered Saline |
PCR: | Polymerase Chain Reaction |
RT: | Reverse Transcription |
SB: | Sleeping Beauty Transposon/Transposase |
Sox2: | SRY (sex determining region Y)-box 2 |
Sox17: | SRY (sex determining region Y)-box 17 |
c-myc: | V-Myc Avian Myelocytomatosis Viral Oncogene Homolog |
UTF1: | Undifferentiated Embryonic Cell Transcription Factor 1 |
References
- Slack JM. Origin of stem cells in organogenesis. Science. 2008;322(5907):1498-501.
- Jones DL, Wagers AJ. No place like home: anatomy and function of the stem cell niche. Nat Rev Mol Cell Biol. 2008;9(1):11-21.
- Spradling A, Drummond-Barbosa D, Kai T. Stem cells find their niche. Nature.2001;414(6859):98-104.
- Sell S. Stem cell origin of cancer and differentiation therapy. Crit Rev Oncol Hematol. 2004 ;51(1):1-28.
- Scadden DT. The stem-cell niche as an entity of action. Nature. 2006;441(7097):1075-9.
- Moore KA, Lemischka IR. Stem cells and their niches. Science. 2006;311(5769):1880-5.
- Tropepe V, Turksen K. The ontogeny of somatic stem cells. Stem Cell Rev. 2012;8(2):548-50.
- Cheung TH, Rando TA. Molecular regulation of stem cell quiescence. Nat Rev Mol Cell Biol. 2013;14(6):329-40.
- Clevers H, Loh KM, Nusse R. Stem cell signaling. An integral program for tissue renewal and regeneration: Wnt signaling and stem cell control. Science.2014;346(6205):1248012.
- Tang DG. Understanding cancer stem cell heterogeneity and plasticity. Cell Res. 2012;22(3):457-72.
- Li L, Clevers H. Coexistence of quiescent and active adult stem cells in mammals. Science. 2010;327(5965):542-5.
- Takeda N, Jain R, LeBoeuf MR, Wang Q, Lu MM, Epstein JA. Interconversion between intestinal stem cell populations in distinct niches. Science. 2011;334(6061):1420-4.
- Blau HM, Brazelton TR, Weimann JM. The evolving concept of a stem cell: entity or function? Cell. 2001;105(7):829-41.
- Clevers H. STEM CELLS. What is an adult stem cell? Science. 2015;350(6266):1319-20.
- Orkin SH, Zon LI. Hematopoiesis: an evolving paradigm for stem cell biology.Cell. 2008;132(4):631-44.
- Phillips RL, Ernst RE, Brunk B, Ivanova N, Mahan MA, Deanehan JK, Moore KA, Overton GC, Lemischka IR. The genetic program of hematopoietic stem cells. Science. 2000;288(5471):1635-40.
- Ding L, Morrison SJ. Haematopoietic stem cells and early lymphoid progenitors occupy distinct bone marrow niches. Nature. 2013;495(7440):231-5.
- Yokota T, Oritani K, Butz S, Kokame K, Kincade PW, Miyata T, Vestweber D, Kanakura Y. The endothelial antigen ESAM marks primitive hematopoietic progenitors throughout life in mice. Blood. 2009;113(13):2914-23.
- Morrison SJ, Spradling AC. Stem cells and niches: mechanisms that promote stem cell maintenance throughout life. Cell. 2008;132(4):598-611.
- Seita J, Weissman IL. Hematopoietic stem cell: self-renewal versus differentiation. Wiley Interdiscip Rev Syst Biol Med. 2010;2(6):640-53.
- Rieger MA, Hoppe PS, Smejkal BM, Eitelhuber AC, Schroeder T. Hematopoietic cytokines can instruct lineage choice. Science. 2009;325(5937):217-8.
- Ding L, Saunders TL, Enikolopov G, Morrison SJ. Endothelial and perivascular cells maintain haematopoietic stem cells. Nature. 2012;481(7382):457-62.
- Tweedell KS. The urodele limb regeneration blastema: the cell potential. ScientificWorldJournal. 2010;10:954-71.
- Cabezas-Wallscheid N, Trumpp A. STEM CELLS. Potency finds its niches. Science. 2016;351(6269):126-7.
- Khan JA, Mendelson A, Kunisaki Y, Birbrair A, Kou Y, Arnal-Estapé A, Pinho S, Ciero P, Nakahara F, Ma'ayan A, Bergman A, Merad M, Frenette PS. Fetal liver hematopoietic stem cell niches associate with portal vessels. Science. 2016;351(6269):176-80.
- Notta F, Zandi S, Takayama N, Dobson S, Gan OI, Wilson G, Kaufmann KB, McLeod J, Laurenti E, Dunant CF, McPherson JD, Stein LD, Dror Y, Dick JE. Distinct routes of lineage development reshape the human blood hierarchy across ontogeny. Science. 2016 Jan 8;351(6269):aab2116.
- Paul F, Arkin Y, Giladi A, Jaitin DA, Kenigsberg E, Keren-Shaul H, Winter D, Lara-Astiaso D, Gury M, Weiner A, David E, Cohen N, Lauridsen FK, Haas S, Schlitzer A, Mildner A, Ginhoux F, Jung S, Trumpp A, Porse BT, Tanay A, Amit I. Transcriptional Heterogeneity and Lineage Commitment in Myeloid Progenitors. Cell. 2015 ;163(7):1663-77.
- Gage FH. Mammalian neural stem cells. Science. 2000;287(5457):1433-8.
- Doetsch F, Caillé I, Lim DA, García-Verdugo JM, Alvarez-Buylla A.Subventricular zone astrocytes are neural stem cells in the adult mammalian brain. Cell. 1999;97(6):703-16.
- Götz M, Huttner WB. The cell biology of neurogenesis. Nat Rev Mol Cell Biol. 2005;6(10):777-88.
- Anthony TE, Klein C, Fishell G, Heintz N. Radial glia serve as neuronal progenitors in all regions of the central nervous system. Neuron. 2004;41(6):881-90.
- Alvarez-Buylla A, García-Verdugo JM, Tramontin AD. A unified hypothesis on the lineage of neural stem cells. Nat Rev Neurosci. 2001;2(4):287-93.
- Ihrie RA, Alvarez-Buylla A. Cells in the astroglial lineage are neural stem cells. Cell Tissue Res. 2008;331(1):179-91.
- Kriegstein A, Alvarez-Buylla A. The glial nature of embryonic and adult neural stem cells. Annu Rev Neurosci. 2009;32:149-84.
- Grabel L. Developmental origin of neural stem cells: the glial cell that could. Stem Cell Rev. 2012;8(2):577-85.
- Lin R, Iacovitti L. Classic and novel stem cell niches in brain homeostasis and repair. Brain Res. 2015;1628(Pt B):327-42.
- Ottone C, Krusche B, Whitby A, Clements M, Quadrato G, Pitulescu ME, Adams RH, Parrinello S. Direct cell-cell contact with the vascular niche maintains quiescent neural stem cells. Nat Cell Biol. 2014;16(11):1045-56.
- Kawaguchi D, Furutachi S, Kawai H, Hozumi K, Gotoh Y. Dll1 maintains quiescence of adult neural stem cells and segregates asymmetrically during mitosis. Nat Commun. 2013;4:1880.
- 39. Jones KM, Sarić N, Russell JP, Andoniadou CL, Scambler PJ, Basson MA. CHD7 maintains neural stem cell quiescence and prevents premature stem cell depletion in the adult hippocampus. Stem Cells. 2015;33(1):196-210.
- Silva-Vargas V, Crouch EE, Doetsch F. Adult neural stem cells and their niche: a dynamic duo during homeostasis, regeneration, and aging. Curr Opin Neurobiol. 2013;23(6):935-42.
- Riquelme PA, Drapeau E, Doetsch F. Brain micro-ecologies: neural stem cell niches in the adult mammalian brain. Philos Trans R Soc Lond B Biol Sci. 2008 ;363(1489):123-37.
- Fuentealba LC, Obernier K, Alvarez-Buylla A. Adult neural stem cells bridge their niche. Cell Stem Cell. 2012;10(6):698-708.
- Tong CK, Chen J, Cebrián-Silla A, Mirzadeh Z, Obernier K, Guinto CD, Tecott LH, García-Verdugo JM, Kriegstein A, Alvarez-Buylla A. Axonal control of the adult neural stem cell niche. Cell Stem Cell. 2014;14(4):500-11.
- Dupin E, Real C, Glavieux-Pardanaud C, Vaigot P, Le Douarin NM. Reversal of developmental restrictions in neural crest lineages: transition from Schwann cells to glial-melanocytic precursors in vitro. Proc Natl Acad Sci U S A. 2003;100(9):5229-33.
- Jessen KR, Mirsky R. The origin and development of glial cells in peripheral nerves. Nat Rev Neurosci. 2005;6(9):671-82.
- Takahashi Y, Sipp D, Enomoto H. Tissue interactions in neural crest cell development and disease. Science. 2013;341(6148):860-3.
- Masaki T, Qu J, Cholewa-Waclaw J, Burr K, Raaum R, Rambukkana A. Reprogramming adult Schwann cells to stem cell-like cells by leprosy bacilli promotes dissemination of infection. Cell. 2013;152(1-2):51-67.
- Xin L, Ide H, Kim Y, Dubey P, Witte ON. In vivo regeneration of murine prostate from dissociated cellpopulations of postnatal epithelia and urogenital sinus mesenchyme . Proc Natl Acad Sci U S A. 2003; 100: (Suppl 1) 11896-903.
- Xin L, Lukacs RU, Lawson DA, Cheng D, Witte ON. Self-renewal and multilineage differentiation in vitro from murine prostate stem cells. Stem Cells. 2007;25(11):2760-9.
- Burger PE, Xiong X, Coetzee S, Salm SN, Moscatelli D, Goto K, Wilson EL. Sca-1 expression identifies stem cells in the proximal region of prostatic ducts with high capacity to reconstitute prostatic tissue. Proc Natl Acad Sci U S A. 2005;102(20):7180-5.
- Leong KG, Wang BE, Johnson L, Gao WQ. Generation of a prostate from a single adult stem cell. Nature. 2008;456(7223):804-8.
- Lawson DA, Xin L, Lukacs RU, Cheng D, Witte ON. Isolation and functional characterization of murine prostate stem cells. Proc Natl Acad Sci U S A. 2007 ;104(1):181-6.
- Richardson GD, Robson CN, Lang SH, Neal DE, Maitland NJ, Collins AT. CD133, a novel marker for human prostatic epithelial stem cells. J Cell Sci. 2004 ;117(Pt 16):3539-45.
- Garraway IP, Sun W, Tran CP, Perner S, Zhang B, Goldstein AS, Hahm SA, Haider M, Head CS, Reiter RE, Rubin MA, Witte ON. Human prostate sphere-forming cells represent a subset of basal epithelial cells capable of glandular regeneration in vivo. Prostate. 2010;70(5):491-501.
- Goldstein AS, Stoyanova T, Witte ON. Primitive origins of prostate cancer: in vivo evidence for prostate-regenerating cells and prostate cancer-initiating cells. Mol Oncol. 2010;4(5):385-96.
- Ousset M, Van Keymeulen A, Bouvencourt G, Sharma N, Achouri Y, Simons BD, Blanpain C. Multipotent and unipotent progenitors contribute to prostate postnatal development. Nat Cell Biol. 2012;14(11):1131-8.
- Shahi P, Seethammagari MR, Valdez JM, Xin L, Spencer DM. Wnt and Notch pathways have interrelated opposing roles on prostate progenitor cell proliferation and differentiation. Stem Cells. 2011;29(4):678-88.
- Luo W, Rodriguez M, Valdez JM, Zhu X, Tan K, Li D, Siwko S, Xin L, Liu M. Lgr4 is a key regulator of prostate development and prostate stem cell differentiation. Stem Cells. 2013;31(11):2492-505.
- Strand DW, Goldstein AS. The many ways to make a luminal cell and a prostate cancer cell. Endocr Relat Cancer. 2015;22(6):T187-97.
- Faraldo MM, Taddei-De La Hosseraye I, Teulière J, Deugnier MA, Moumen M, Thiery JP, Glukhova MA. [Mammary gland development: Role of basal myoepithelial cells]. J Soc Biol. 2006;200(2):193-8.
- Petersen OW, Polyak K. Stem cells in the human breast. Cold Spring Harb Perspect Biol. 2010;2(5):a003160.
- Shackleton M, Vaillant F, Simpson KJ, Stingl J, Smyth GK, Asselin-Labat ML, Wu L, Lindeman GJ, Visvader JE. Generation of a functional mammary gland from a single stem cell. Nature. 2006;439(7072):84-8.
- Wang D, Cai C, Dong X, Yu QC, Zhang XO, Yang L, Zeng YA. Identification of multipotent mammary stem cells by protein C receptor expression. Nature. 2015 ;517(7532):81-4.
- Van Keymeulen A, Rocha AS, Ousset M, Beck B, Bouvencourt G, Rock J, Sharma N, Dekoninck S, Blanpain C. Distinct stem cells contribute to mammary gland development and maintenance. Nature. 2011;479(7372):189-93.
- Hassiotou F, Beltran A, Chetwynd E, Stuebe AM, Twigger AJ, Metzger P, Trengove N, Lai CT, Filgueira L, Blancafort P, Hartmann PE. Breastmilk is a novel source of stem cells with multilineage differentiation potential. Stem Cells. 2012;30(10):2164-74.
- Rios AC, Fu NY, Lindeman GJ, Visvader JE. In situ identification of bipotent stem cells in the mammary gland. Nature. 2014;506(7488):322-7.
- LaBarge MA, Nelson CM, Villadsen R, Fridriksdottir A, Ruth JR, Stampfer MR, Petersen OW, Bissell MJ. Human mammary progenitor cell fate decisions are products of interactions with combinatorial microenvironments. Integr Biol (Camb). 2009;1(1):70-9.
- LaBarge MA. On stem cells in the human breast. Cold Spring Harb Perspect Biol. 2012;4(5). pii: a013441.
- Mishra L, Shetty K, Tang Y, Stuart A, Byers SW. The role of TGF-beta and Wnt signaling in gastrointestinal stem cells and cancer. Oncogene. 2005;24(37):5775-89.
- Medema JP, Vermeulen L. Microenvironmental regulation of stem cells in intestinal homeostasis and cancer. Nature. 2011;474(7351):318-26.
- Cheng H, Leblond CP. Origin, differentiation and renewal of the four main epithelial cell types in the mouse small intestine. V. Unitarian Theory of the origin of the four epithelial cell types. Am J Anat. 1974;141(4):537-61.
- Clevers H. The intestinal crypt, a prototype stem cell compartment. Cell. 2013;154(2):274-84.
- Clevers H, Batlle E. SnapShot: the intestinal crypt. Cell. 2013;152(5):1198-1198.e2.
- Clevers H. Stem Cells: A unifying theory for the crypt. Nature. 2013;495(7439):53-4.
- Barker N, van de Wetering M, Clevers H. The intestinal stem cell. Genes Dev. 2008;22(14):1856-64.
- Potten CS. Extreme sensitivity of some intestinal crypt cells to X and gamma irradiation. Nature. 1977;269(5628):518-21.
- Li VS, Clevers H. In vitro expansion and transplantation of intestinal crypt stem cells. Gastroenterology. 2012;143(1):30-4.
- Sangiorgi E, Capecchi MR. Bmi1 is expressed in vivo in intestinal stem cells. Nat Genet. 2008;40(7):915-20.
- Buczacki SJ, Zecchini HI, Nicholson AM, Russell R, Vermeulen L, Kemp R, Winton DJ. Intestinal label-retaining cells are secretory precursors expressing Lgr5. Nature. 2013;495(7439):65-9.
- van Es JH, Sato T, van de Wetering M, Lyubimova A, Nee AN, Gregorieff A, Sasaki N, Zeinstra L, van den Born M, Korving J, Martens AC, Barker N, van Oudenaarden A, Clevers H. Dll1+ secretory progenitor cells revert to stem cells upon crypt damage. Nat Cell Biol. 2012;14(10):1099-104.
- Barker N. Adult intestinal stem cells: critical drivers of epithelial homeostasis and regeneration. Nat Rev Mol Cell Biol. 2014;15(1):19-33.
- Carulli AJ, Samuelson LC, Schnell S. Unraveling intestinal stem cell behavior with models of crypt dynamics. Integr Biol (Camb). 2014;6(3):243-57.
- Yan KS, Chia LA, Li X, Ootani A, Su J, Lee JY, Su N, Luo Y, Heilshorn SC, Amieva MR, Sangiorgi E, Capecchi MR, Kuo CJ. The intestinal stem cell markers Bmi1 and Lgr5 identify two functionally distinct populations. Proc Natl Acad Sci U S A. 2012;109(2):466-71.
- Visvader JE, Clevers H. Tissue-specific designs of stem cell hierarchies. Nat Cell Biol. 2016;18(4):349-55.
- Booth BW, Mack DL, Androutsellis-Theotokis A, McKay RD, Boulanger CA, Smith GH. The mammary microenvironment alters the differentiation repertoire of neural stem cells. Proc Natl Acad Sci U S A. 2008;105(39):14891-6.
- Boulanger CA, Mack DL, Booth BW, Smith GH. Interaction with the mammary microenvironment redirects spermatogenic cell fate in vivo. Proc Natl Acad Sci U S A. 2007;104(10):3871-6.
Corresponding Author
- Stoyan Petkov, German Primate Center, Kellnerweg 4,37077 Göttingen, Germany; Email: spetkov@dpz.eu
- Heiner Niemann, Institute for Farm Animal Genetics, Friedrich-Loeffler-Institute, Hoeltystrasse 10, 31535 Neustadt am Ruebenberge, Germany; Email: heiner.niemann@fli.bund.de